Joining forces with biology to close the critical mineral gap
by Dr. Isabella Fandrych, General Partner at Nucleus Capital. | Originally published on Medium.
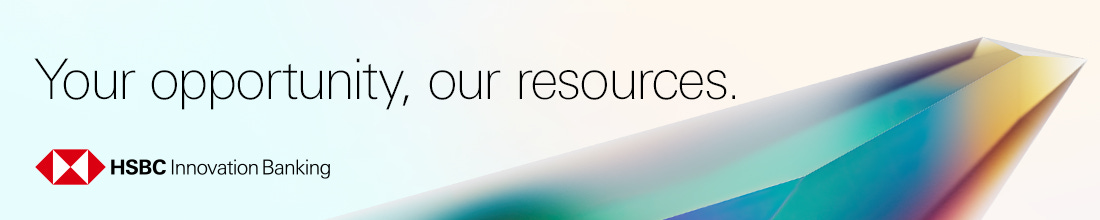
Guest post by Dr. Isabella Fandrych, General Partner at Nucleus Capital. | Originally published on Medium.
This article is the second in a series exploring the future of mining in the context of the global energy transition. The first part, Energy Transition at Risk: The Imperative to Innovate in Mining, examined the risk that the substantial supply gap of critical minerals poses for the expansion of renewable energy infrastructure and, consequently, for decarbonization in the face of accelerating climate change. In this article, we explore methods for extracting critical minerals through recycling and mining. We are particularly interested in approaches leveraging previously untapped critical mineral deposits, e.g., in lithium and copper extraction, and how biology can help navigate scarcities and supply constraints.
Demand cannot be solved with recycling alone.
As discussed in our previous article, we anticipate a significant growth in demand for critical minerals.
Globally, players like Cylib, Redwood Materials, and Ascend Elements are looking to recover minerals from secondary sources. While the infrastructure and supply chains for metals such as gold, silver, and platinum are well-established, the recovery rate for critical minerals remains exceedingly low (Figure 1). Substantial barriers continue to obstruct a transformative shift in this domain:
Rare Earth Elements (REEs) are frequently alloyed with other minerals in products such as magnets and touchscreens. Separating these elements from composite materials traditionally involves hazardous chemicals and high energy intensity. Procuring these elements from primary sources often remains more cost-effective than recovering them from secondary materials².
Moreover, the availability of secondary materials is currently limited, as only a small proportion of electric vehicles (EVs) and batteries have reached the end of their lifecycle. Decades will pass before sizeable volumes of spent battery metals become available for recycling, with major quantities expected only after 203⁰². The World Bank estimates that even in an optimistic scenario, where a 100% recycling rate for critical metals is achieved by 2050, a notable supply gap will persist⁴. Increasing primary supply capacities is therefore indispensable.
Traditional extraction methods are insufficient.
The need for increasing primary supply is particularly critical in the case of lithium and copper. To understand how we can increase output in these minerals, it is important to understand the limitations of extraction approaches used today. In this section, we will highlight mining approaches and challenges in lithium and copper.
Lithium is mainly extracted by mining ores from hard rock or via evaporation ponds of lithium-containing brines.
Hard rock mining is the more prevalent method, accounting for approximately 60% of global lithium production⁵. This approach involves processing lithium-rich ores through energy-intensive methods such as acid roasting, which requires high temperatures and concentrated acids. Although hard rock mining projects generally have shorter development and processing times compared to brine-based methods, they are associated with significant environmental impact, including higher CO₂ emissions and lower profit margins⁶.
Brine evaporation is emerging as an increasingly important source, given that the majority of the world’s lithium reserves are contained in brines. One of the challenges is that lithium concentrations in brines are often lower and mixed with other ions such as magnesium, calcium, and sodium, necessitating complex extraction processes. To produce lithium compounds incorporated into batteries, such as lithium carbonate (Li₂CO₃) or lithium hydroxide (LiOH), it is necessary to selectively separate lithium from other ions in the brine. Conventional brine operations pump lithium-rich brine to the surface, where it is concentrated through solar evaporation over months to years⁶ ⁷. This process, while less energy-intensive, is slow and results in noteworthy lithium losses (over 40%), along with a hefty environmental footprint due to water and land usage, and potential toxic leakages tied to acid usage⁶. Consequently, the challenges posed by traditional lithium extraction methods have led to a growing interest in more techno-economically viable and environmentally friendly alternatives to evaporation.
In light of these challenges, innovators have developed approaches to selectively isolate lithium from brine. Direct Lithium Extraction (DLE) methods offer a paradigm shift in lithium extraction by increasing brine recovery rates to 70–90%, reducing extraction times from months to days⁶. Currently, the most advanced approaches in DLE include adsorption, ion exchange, solvent extraction, the utilization of membranes, and electrochemical methods. An overview of these methods is provided in the table below² ⁶ ⁷.
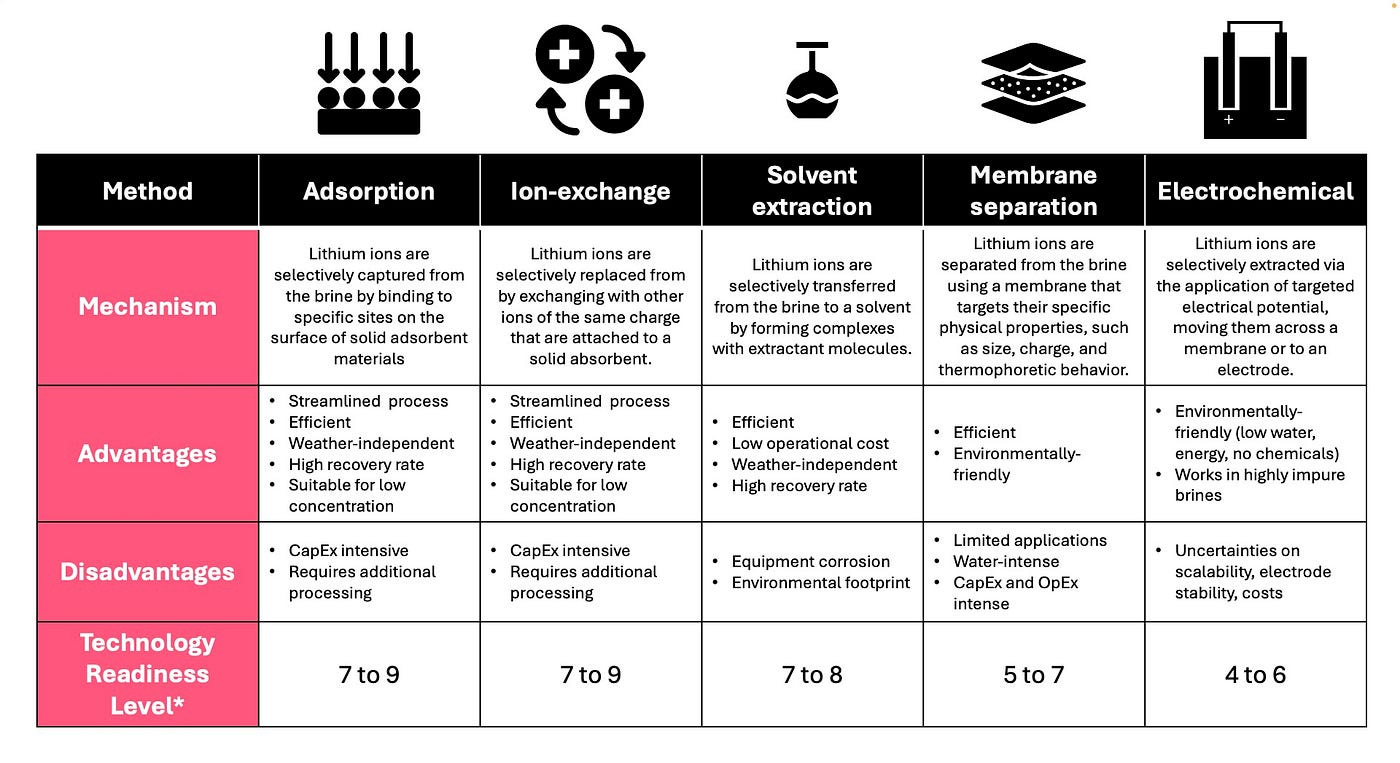
One of the downsides of DLE includes high energy intensity, one of the most vital metrics, and at the same time one of the biggest challenges, is the degree of selectivity of the method — i.e., the ability of the extraction process to preferentially isolate lithium ions over other competing ions, such as magnesium (Mg), sodium (Na), and calcium (Ca), which are commonly present in brine solutions. Without sufficient selectivity, brines with high ratios of Mg or Na can become economically unfeasible to mine due to the extensive and costly additional steps required to separate lithium from these competing ions. For instance, ion exchange resins with a Li/Mg selectivity ratio of around 10 mean that for every 10 lithium ions extracted, a magnesium ion may also be captured, leading to impure outputs and the need for additional purification steps.
Similar challenges can be observed in the case of copper. Humanity has had an insatiable appetite for this metal since the dawn of civilization, and this demand is set to grow substantially in the face of global electrification ambitions. However, copper reserves are finite, with the majority of high-grade copper ore already depleted. 70% of the remaining copper reserves are trapped in low-grade ores such as chalcopyrite, with concentrations as low as 10%⁸. These vast deposits, bound in complex chemical structures, are significantly more difficult to extract economically with current copper extraction methods, namely pyrometallurgy and hydrometallurgy⁹.
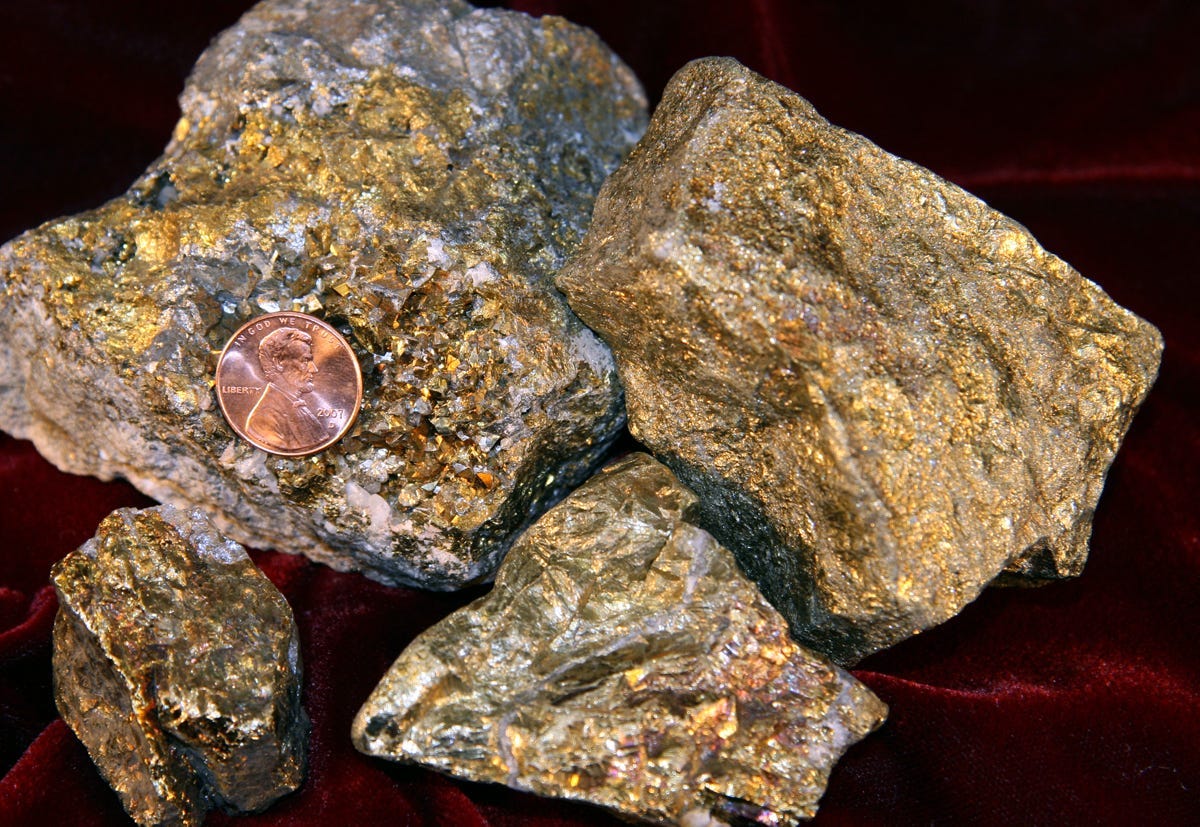
Pyrometallurgy, the most common method for extracting copper, involves heating ores to high temperatures to separate the metal from other elements. While effective for high-grade ores, this method is inefficient for low-grade chalcopyrite, which contains minimal copper content, making direct smelting inefficient and costly.
Hydrometallurgical methods employ chemical solutions, known as lixiviants, to leach target metals out of the ore. Yet, due to its high iron content, chalcopyrite is classified as a refractory mineral, meaning it exhibits profound resistance to decomposition by heat or chemical processes. Consequently, it requires elevated temperatures and oxidizing conditions to effectively release its copper into the solution. As ore concentrations decrease, the increased energy and chemical requirements make the process progressively less economically viable.
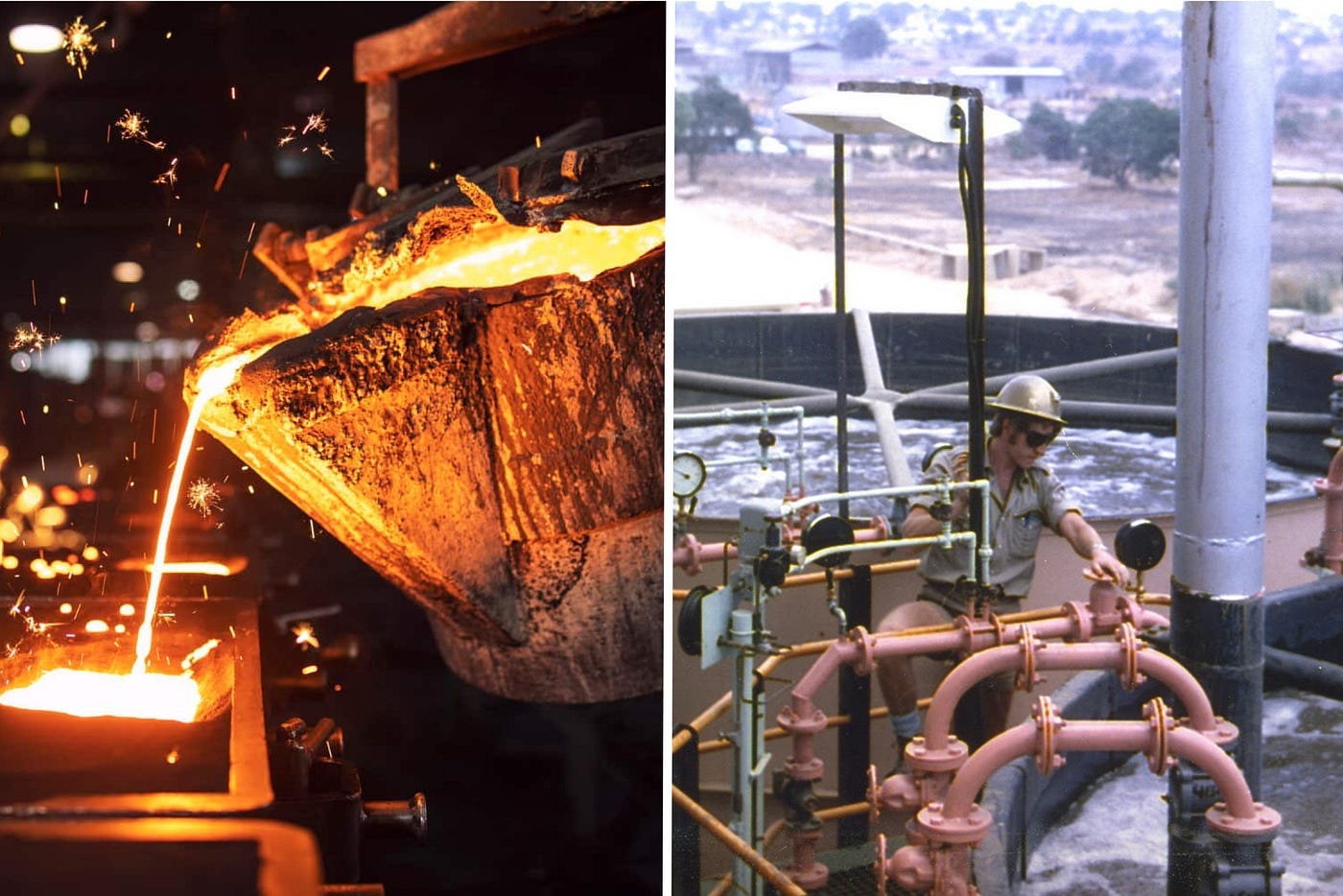
Amidst the urgent need for primary copper production to enable global electrification, viable methods for extracting the required metal deposits remain elusive — posing a considerable challenge for accessing the minerals so critically needed to decarbonize energy systems.
Closing the gap with nature.
At Nucleus Capital, our thesis is centered around a technology field that has seen millions of years of research: biology. As with fermentation in food preservation, humans have unknowingly utilized biological processes for millennia in the context of metal production, a practice now termed bio-mining (or, more broadly, biohydrometallurgy). Documents suggest that as early as 7th-century BC China¹⁰, the oxidative capabilities of bacteria were inadvertently employed in copper production, allowing the country to scale its output significantly. It was not until much later, in 1951, that the scientific foundation for our contemporary understanding of metals in metabolic pathways was established in a paper by Kenneth Temple and Arthur Colmer¹¹.
Today, in the era of programmable biology, the concept of bio-mining can be reinvented, and the pathways and tools utilized by bacteria, fungi, and plants can be adapted to extract and refine the metals essential for expanding renewable energy infrastructure.
Among the recent discoveries in the field is an extremophile microbe found in high-stress environments, which relies on a specific set of rare earth elements for its metabolic processes. Given the scarce concentration of these elements, evolution — arguably the universe’s most prolific R&D department — has equipped this microbe with a protein that exhibits picomolar affinity, binding at concentrations as low as one trillionth (10⁻¹²) of a mole per liter, with an unprecedented level of selectivity for these elements.
Employing synthetic biology’s toolkit, such a protein could be engineered to selectively bind with target metals such as lithium. Utilizing a bioengineered version of this protein with unprecedented selectivity could enable extraction from brines currently deemed too contaminated. As a result, complementing current DLE methods with biochemical mechanisms could significantly reduce the required processing steps, energy, heat, harmful chemicals, and equipment. This, in turn, reduces operational costs and enhances the overall profitability of lithium extraction, particularly for brines heavily contaminated with Mg and Na — potentially enabling the economic viability of previously unfeasible mining sites.
Bio-mining also has received attention in other ores such as copper and gold. For example, companies leverage microbes to extract copper from high-impurity ores. Their microbial communities are capable of facilitating the extraction process from low-grade deposits to previously unseen techno-economics through bio-leaching, as the chemicals they naturally produce serve as effective bio-lixiviants to break down chalcopyrite. Leveraging microbes to help miners increase their yields brings the environmental benefits of adding less heat, energy, and acids to the mining process. Programming biology might, therefore, unlock copper resources previously deemed too energy-intensive and expensive to mine.
Introducing (synthetic) biology into the world of mining could represent a paradigm shift for the clean energy transition, enabling the access and refinement of necessary minerals across a range of novel deposits with diversified supply chains, increased profitability, and enhanced environmental efficiency.
At Nucleus Capital, we are particularly excited about use cases with the potential to alleviate critical mineral shortages essential for energy system decarbonization. Simultaneously, the (synthetic) biology toolkit allows for the exploration of a wide array of applications, extending to other target materials and use cases, such as the separation of metals from waste or the remediation of contaminated environments (bio-remediation).
On creating symbioses with the mining industry
While technological innovation in the form of synthetic biology holds the potential for a transformative shift within the industry, numerous hurdles must be navigated to achieve broad adoption. Startups, in particular, must prove themselves among established mining incumbents. The mining industry imposes especially high standards on emerging players.
Large mining projects frequently require hundreds of millions to billions on upfront investment¹³. Operators will not allow the integration into their flowsheet if it has not been extensively proven and de-risked through a series of rigorous tests. Part of this involves anticipating a seamless and versatile integration into established extraction processes. Bio-approaches need to directly integrate into mining facilities without changing existing infrastructure and flowsheets. Easy adoption is also necessary, considering incumbents often have limited experience with biology-based approaches.
Bio-mining entrepreneurs have the unique opportunity to simultaneously drive massive economic and environmental impact. Already today, mining encompasses a trillion-dollar industry, set to grow tremendously in humanity’s never-ending appetite for metals, especially amid the huge demand for clean energy infrastructure.
If you’re a founder working on innovative solutions along the critical mineral supply chain, please reach out to Isabella (isabella@nucleus-capital.com) or submit your deck to our website! Likewise, if you’re a miner interested in connecting with companies at the forefront of mineral extraction using biology, please don’t hesitate to get in touch!
References
International Energy Association. (2021). Growth in demand for selected minerals from clean energy technologies by scenario, 2040 relative to 2020. https://www.iea.org/data-and-statistics/charts/growth-in-demand-for-selected-minerals-from-clean-energy-technologies-by-scenario-2040-relative-to-2020, Licence: CC BY 4.0. Accessed August 22, 2024.
Ferris, N. (2023, March 24). Why recycling is no golden ticket to endless critical minerals. Energy Monitor. https://www.energymonitor.ai/tech/why-recycling-is-no-golden-ticket-to-endless-critical-minerals/. International Energy Association
International Energy Association. (2021). End-of-life recycling rates for selected metals. https://www.iea.org/data-and-statistics/charts/end-of-life-recycling-rates-for-selected-metals, Licence: CC BY 4.0. Accessed August 22, 2024.
Hund, K. L., Arrobas, D. L. P., Fabregas Masllovet, T. P., Laing, T. J., & Drexhage, J. R. (2023). Minerals for climate action: The mineral intensity of the clean energy transition. World Bank Group. http://documents.worldbank.org/curated/en/099052423172525564/P16627806f5aa400508f8c0bdcba0878a3e. Accessed August 22, 2024.
Benchmark Source. (2023). Hard rock lithium vs. brine — How do their carbon curves compare? Benchmark Source. https://source.benchmarkminerals.com/article/hard-rock-vs-brine-how-do-their-carbon-curves-compare. Accessed August 22, 2024.
Goldman Sachs Equity Research (2021). Direct Lithium Extraction: A potential game changing technology. Goldman Sachs. Accessed August 15, 2023.
Werny, M. (2024, January 5). The technology overview: closing the lithium supply gap with direct lithium extraction (DLE) and battery recycling. Extantia Capital. https://medium.com/extantia-capital/the-technology-overview-closing-the-lithium-supply-gap-with-direct-lithium-extraction-dle-and-d686e0e7a923. Accessed August 22, 2024.
S&P Global. (2022). The Future of Copper. https://cdn.ihsmarkit.com/www/pdf/0722/The-Future-of-Copper_Full-Report_14July2022.pdf. Accessed August 22, 2024.
Li, X., Monnens, W., Li, Z., Fransaer, J., & Binnemans, K. (2020). Solvometallurgical process for extraction of copper from chalcopyrite and other sulfidic ore minerals. Green Chemistry, 22(2), 417–426. https://doi.org/10.1039/c9gc02983d. Accessed August 22, 2024.
Qiu, G., Liu, X., Zhang, R. (2023). Biomining in China: History and Current Status. In: Biomining Technologies. https://doi.org/10.1007/978-3-031-05382-5_8. Accessed August 22, 2024.
Temple, K. L., & Colmer, A. R. (1951). The autotrophic oxidation of iron by a new bacterium, thiobacillus ferrooxidans. Journal of Bacteriology, 62(5), 605–611. https://doi.org/10.1128/jb.62.5.605-611.1951. Accessed August 22, 2024.
Reuters. (2019). Game of Mines. https://www.reuters.com/graphics/ELECTRIC-VEHICLES-METALS/010092JB38P/. Accessed August 22, 2024.
Mining Technology. (2023, March 17). Mining capital expenditure to rise by 12% across leading miners in 2023. Mining Technology. https://www.mining-technology.com/analyst-comment/mining-capital-expenditure-2023/. Accessed August 22, 2024.
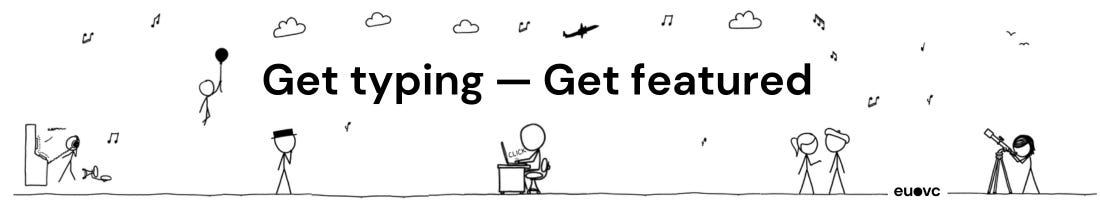